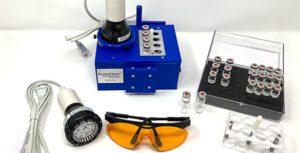
In the event you get inspired to start investigating photochemistry, we offer the above Starter Bundle that includes everything you need, including four sample reactions.
Quick Introduction
Photochemistry is conquering the world of synthetic chemistry. Ok, that’s hyperbole. Photochemistry is really cool and useful for making interesting and important compounds in ways that may be easier, safer or not possible with traditional thermal methods. Ok, that’s better. More specifically, for our purposes we are referring to visible light photoredox catalysis – catalytic reactions initiated in some way by visible light. These days, it is difficult to read a chemical journal without seeing multiple new protocols using visible light as the key reagent in a chemical transformation. Maybe you thought this was a fad, like microwave (we joke) or maybe you just really like your oil bath (weird). However, if you have never put on a pair of fashionable orange glasses, turned on a bright blue LED, and pointed it at a reaction flask, then you are missing out. Photochemistry will bring you hours of joy and millions of dollars (most likely not). In all seriousness, at a minimum, this three part series is intended to get you comfortable with the core principles of photochemistry and provide you with everything you need to know to get started.
If you think you know everything about the basics of photochemistry, you can skip ahead to Photochemistry 101, Part II: Understanding and Measuring Light Sourcesfor a discussion on light sources. If you really, really think you know everything about photochemistry and just want to set up a few reactions, then wait for us to publish Photochemistry 101, Part III: Setting Up Your Initial Photochemistry Reactions. Otherwise, read on and reacquaint yourself with the foundations of photochemistry principles.
Let’s Start with the Basics of Photoredox Catalysis
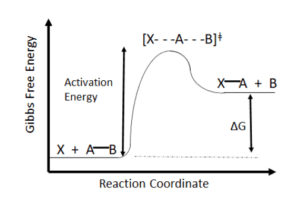
Figure 1: Gibbs Free Energy Diagram
If you are reading this, you are probably a chemist, more likely a synthetic chemist (if not, feel free to keep reading anyway). Chances are, you have assembled and broken a few chemical bonds in your day. Also, chances are pretty good that you have heated a flask at some point in your life. The reason that you did this was to overcome the activation energy for the reaction, the barrier needed for the reaction to occur ( see Figure 1). As the energy in a system increases, there is sufficient energy for the two reagents to come together, make or break the bonds as needed and cross to the other side (Ref 1).
In photoredox catalysis, we want to use a photoactive compound to transfer the energy from light into a chemical energy that can be used to drive a chemical reaction. Photons (light) are energy in its most concentrated form; however, most chemical reactions are unable to access this energy in a useful or controlled way. Activating chemical reactions using light draws on what have been termed the two laws of photochemistry:
- Light must be absorbed by a molecule for the chemical reaction to take place. (Grotthuss-Draper law).
- For each photon of light absorbed by a molecule, only one molecule is activated for a photochemical reaction. (Stark-Einstein law)
Understanding Light vs Heat
Light is generally divided into three classifications, ultraviolet (100 to 380 nm), visible (380-700 nm) and infrared (greater than 700 nm). The amount of energy in light is highest at shorter wavelengths and much lower at longer wavelengths. Ultraviolet light is strong enough to directly break chemical bonds and initiate free radical reactions and radical rearrangements. For synthetic purposes, think classical reactions such as [2+2] photocycloadditions of α,β-unsaturated ketones to alkenes. This high energy; however, makes functional group tolerance very low. UV has enough energy to directly damage DNA and give you a bad sunburn but does not penetrate your skin deeply.
Infrared is the weakest, invisible to the human eye and accounts for what is generally perceived as “heat radiation”. Infrared radiation is emitted and absorbed by molecules during rotations and vibrations and useful chemically for isomerizations, cleavage reactions and activation of prodrugs in vivo (Ref 2). Red light can travel the farthest through materials, passing through your skin with minimal absorbance.
Between these two extremes is visible light (you guessed it, the light you can see with your naked eye). Visible light accounts for nearly all of the energy on earth used by living things, once it has been converted to carbohydrates via photosynthesis. Lucky for us, most of the chemicals around us don’t absorb much in the visible region and when they do, not much happens. In general, most things are fairly inert to visible light. Otherwise, there might be a little too much reactivity going on in your morning cup of coffee and the shelf-life of your bottle of Tylenol might be pretty short.
What Makes a Good Photocatalyst
Getting back to our reaction diagram, in order to access the energy visible-from light chemically, the photon needs to be absorbed by something in our reaction. Often, we need a specific type of molecule with strong absorbance bands in the visible region, for example highly conjugated organic dyes or metal complexes with bulky aromatic ligands. Compounds that are excited by light and can transfer the energy to something else are referred to as photosensitizers. For our purposes, these are our photocatalysts.
So what makes a good photocatalyst? At minimum, we need a catalyst that can absorb light in the visible region (where most are substrates are inert), resulting in an excited state. We need this excited state to live long enough to undergo bimolecular reactions (with our substrates). Often, the catalyst in the ground state is a poor single electron oxidant or reductant (otherwise they might undergo unwanted reactions); however, upon excitation by light give a powerful single-electron transfer agent. Additionally, the catalyst should be stable to the chemistry you want to activate and have no other interactions with the chemistry that you are doing (unless its productive, then of course it is advantageous)
A large number of well-characterized commercially available photocatalysts exist due to their extensive use in renewable energy, imaging and biological applications (Ref 3). Most common are organometallic photocatalysts, many relying on ruthenium and iridium metals with polypyridyl ligands. Alternately, organic dyes like MesAcr and Eosin Y are efficient photocatalysts. Sometimes you may not even need a catalyst (Ref 4). For fear of leaving out a discussion of someone’s favorite catalyst (send us your favorite catalyst @EvoluChem on twitter), here are a few of the most common photocatalysts available (see Figure 2 below).
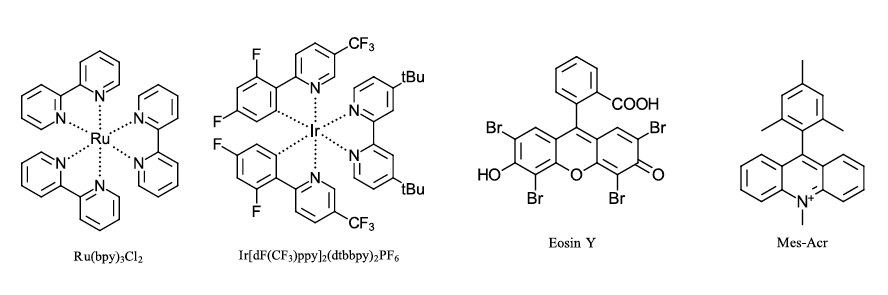
Figure 2: Frequently used catalysts for photoredox transformations.
Excited States and Activating Difficult Transformations
For most photochemistry, there are three important electronic states to consider for the photocatalyst, S0, S1, and T1 (see Figure 3). When a molecule in its ground state (S0) absorbs one photon of light, it causes a single electron to be excited to a higher molecular orbital (S1). Based on spin selection rules this electron maintains its spin in the new orbital in a singlet state. Multiple singlet states are possible at increasing energy levels; however, the high energy nature of these singlets suggests that they should quickly relax by decay or internal conversion back to S1. Unproductively for chemical transformations, S1 can relax back to S0 via internal conversion with energy loss as heat or can also give off a photon via fluorescence.
Important to its role as a “catalyst”, alternatively S1 can undergo intersystem crossing (ISC) with spin inversion to generate an excited triplet state T1. This new T1 state should be both lower in energy and exist with a longer lifetime than the S1 state, and thus can exist with sufficient lifetime to undergo bimolecular reactions via energy transfer (EnT) or electron transfer (ET). Unproductively, T1 can relax back to S0 via either internal conversion or by emitting a photon via phosphorescence.
This new T1 state, with a lifetime ranging from micro to milliseconds, exists with two interesting features. By the nature of a half-filled low-energy orbital the excited state catalyst is significantly more oxidizing than the ground state catalyst. Simultaneously, the T1 state has an unpaired election in a high energy orbital and can act as a strong reductant as well. This unique feature of excited photocatalysts, the ability to give or receive an electron as needed, opening up numerous possibilities for novel catalytic cycles with the excited photocatalyst present to regulate oxidative and reductive steps as needed.
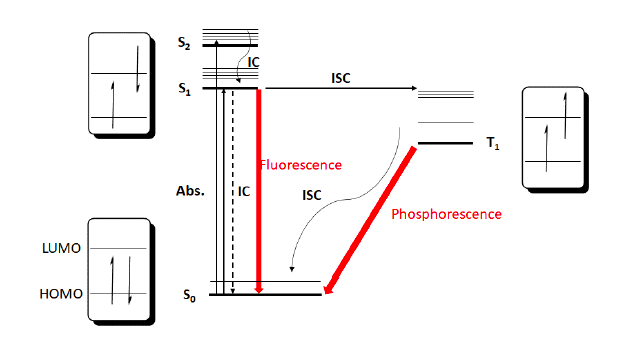
Figure 3: Jablonski plot for excitation of a photocatalyst
Ru(bpy)3 is the most widely used photocatalyst with well-established photochemical properties suitable for photoredox catalysis (Ref 5). First, with a strong absorbance maximum ~450 nm in the visible region, Ru(bpy)32+ can be excited with fluorescent light bulbs, LEDs, Xe lamps and sunlight (Figure 4a). The resulting *[Ru(bpy)3]2+ triplet state has a significant lifetime (1 μs), long enough to undergo single electron transfer (SET) to organic molecules. The excited state can either proceed through a reductive quenching cycle (Figure 4b, c) or oxidative quenching cycle (Figure 4d, e) acting as both 1 e– oxidant and 1 e– reductant generating open shell reactive species (A·- or D·+).
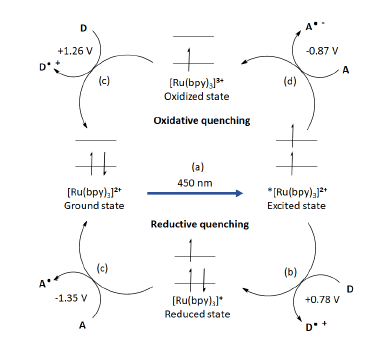
Figure 4: Excited state of Ru(bpy)3 leading to an oxidative and reductive quenching cycles (Figure adapted from Ref 5)
It is no surprise that the well-established catalytic cycle for Ru(bpy)3 was used for the photoredox reactions that kicked off interest in this field (see Figure 5). Starting in 2008, initial reports by 3 groups with three diverse reactions, a.) 2+2 cycloaddition (Ref 6), b.) dehalogenation (Ref 7) and c.) organocatalyzed alkylation of aldehydes (Ref 8) demonstrate the early promise of light as the reagent. After these 3 reports, chemists everywhere repurposed their grow lights (sorry “aquarium bulbs”), flashlights and LED strips, built some home-made reactors and started discovering some incredible useful chemical transformations.
In the following 12 years, the number of useful reactions fills multiple reviews and is too vast to discuss here in depth. You can read about metallophotoredox (Ref 9), organic dyes (Ref 10), the impact of photoredox on synthesis (Ref 11) and reactions in flow (Ref 12) among others. A nearly unlimited number of photosensitizing compounds are commercially available to try, while new catalyst design continues to push the boundaries of what is possible (such as the recent demonstration by Miyake of the photochemical Birch reaction (Ref 13), However, perhaps most relevant for many medicinal chemists is best described by MacMillan and coworkers combining an Iridium photoredox cycle to activate nickel catalyzed organometallic cycles (see Figure 6) (Ref 14). This reaction paradigm, using the photoredox cycle to activate an organometallic cycle, has afforded photoredox versions of many traditional organometallic cross coupling chemistry (C-C, C-N, C-O, C-S coupling) traditionally done thermally with palladium, nickel, copper, catalysts among others (Ref 9)
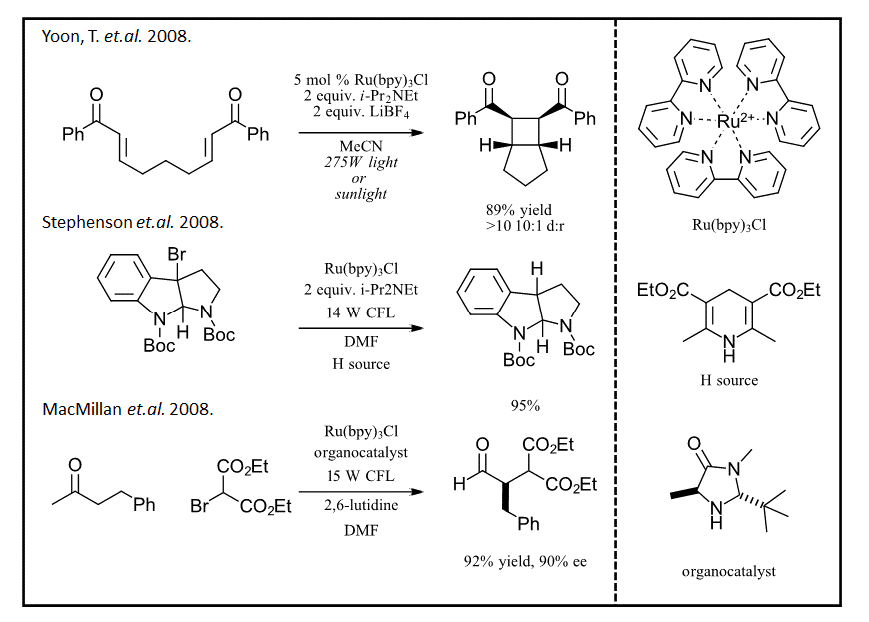
Figure 5: Photoredox renaissance beginning in 2008 (Ref 6-8)
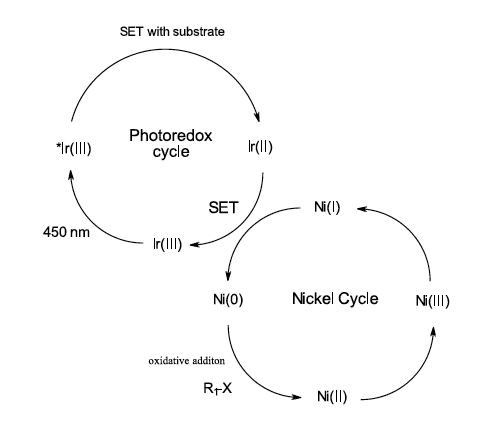
Figure 6: Photoredox activation of organometallic cycle
We’ll go into further detail on a few useful visible light catalyzed reactions in Photochemistry 101: Part III (coming soon!), but over the past decade photochemical parallels to many traditional “named” organometallic cross coupling reactions have been discovered. Once we can convert visible light into chemical energy, photoredox catalysis enables chemical transformations with several potential beneficial features over a corresponding thermal reaction:
- Room temperature compared to often high temperatures
- Lower catalyst loadings
- Facile access to open shell reactive species without the need for stoichiometric oxidants/reductants or radical initiators (for example, eliminating toxic tin reagents)
- Activation of sluggish catalytic cycles
- Access to both oxidative and a reductive conditions simultaneously
You just read the first part of a three part series designed to help you get started in photochemistry. Below are links to all three parts of the series. Any questions? Send them to info@hepatochem.com, we’d love to hear from you!
Here’s the entire series:
Photochemistry 101, Part I: Everything You Need To Know To Get Started
Photochemistry 101, Part II: Understanding and Measuring Light Sources
Photochemistry 101, Part III: Setting Up Your Initial Photochemistry Reactions
References
- Yes, this is a simplified explanation, there are entire textbooks written about this stuff.
- https://hepatochem.com/red-light-applications-in-photochemistry/
- Don’t worry, there’s still room for you to synthesize 50 nearly identical derivatives of your favorite chromophore.
- https://hepatochem.com/electron-donor-acceptor-eda-complexes-in-photochemistry/
- Tucker, J. and Stephenson, C. R. J. “Shining Light on Photoredox Catalysis: Theory and Synthetic Applications”, Journal of Organic Chemistry, 2012, 77, 1617-1622.
- Ischay, M. A.; Anzovino, M. E.; Du, J.; Yoon, T. P. J. Am. Chem. Soc. 2008, 130, 12886.
- Narayanam, J. M. R.; Tucker, J. W.; Stephenson, C. R. J. J. Am. Chem. Soc. 2009, 131, 8756.
- Nicewicz, D. A.; MacMillan, D. W. C. Science 2008, 322, 77.
- Shaw, M. H.; Twilton, J.; MacMillan, D. W. C. Photoredox Catalysis in Organic Chemistry. J. Org. Chem. 2016, 81, 6898 ’6926. https://pubs.acs.org/doi/abs/10.1021/acs.joc.6b01449
- Romero, N., Nicewicz, “Organic Photoredox Catalysis”, Chemical Reviews, 2016 (116), 10075-10166.
- Marzo, L.; Pagire, S. K.; Reiser, O.; König , B. Visible light Photocatalysis: Does It Make a Difference in Organic Synthesis? Angew. Chem., Int. Ed. 2018, 57, 10034-10072.
- Harper, K. Moschetta, E., Bordawekar, S., Wittenberger, S. “A Laser Driven Flow Chemistry Platform for Scaling Photochemical Reactions with Visible light., ACS Central Science, 2019 (5), 109-115.
- Justin P. Cole, Dian-Feng Chen, Max Kudisch, Ryan M. Pearson, Chern-Hooi Lim, and Garret M. Miyake, “Organocatalyzed Birch Reduction Driven by Visible light, J. Am. Chem. Soc, 2020, 142, 13573-13581. https://pubs.acs.org/doi/abs/10.1021/jacs.0c05899
- Zuo, Z., Ahneman, D., Chu, L., Terrett, J., Doyle, A., Macmillan, D. “Merging photoredox with nickel catalysis: Coupling of α-carboxyl sp3-carbons with aryl halides” Science, 2014 (345), 437-440.