Multi-photon approaches to synthetic photochemistry expand the potential pathways to create more efficient and potentially safer reaction conditions.Wenger and coworkers recently published a fascinating in-depth review on multi-photon excitation in photoredox catalysis (Ref 1). The general paradigm of most modern synthetic photoredox catalysis involves absorption of one photon of visible light by a photoactive catalyst to give an oxidative/reductive species that can give or receive an electron as needed. Thus, the majority of preparative scale photoredox reactions reported to date invoke catalytic cycles where 1 photon productively absorbed by the photocatalyst is required for 1 equivalent of product. Two factors limit the range of reactivity available via traditional photoredox:
- The energy limit of visible photons (up to 300 kJ/mol) available before transitioning to higher energy UV where many substrates would also absorb.
- The energy loss that occurs between the absorption of photon by the photocatalyst and resulting species required for the activation of a substrate.
Between 25% to 50% of the visible energy absorbed by the photocatalyst is lost before being utilized through internal processes such as Internal conversion, intersystem crossing, or oxidation or reduction of the catalyst. As such, a limited range of redox potentials are available for traditional photoredox catalysts (Table 1).
Table 1: Excited state oxidation and reduction potential limits for several common Iridium and ruthenium photocatalysts (Ref 2)
Catalyst | Oxidation | Reduction | ||
Ir(III)(dF-CF3-ppy)2(dtbpy) | Ir(III)*/Ir(II) | 1.21 V | Ir(III)*/Ir(IV) | -0.89 V |
Ru(II)(phen)3 | Ru(II)*/Ru(I) | 0.82 V | Ru(II)*/Ru(III) | -0.87 V |
Ru(II)(bpy)3 | Ru(II)*/Ru(I) | 0.77 V | Ru(II)*/Ru(III) | -0.81 V |
Ir(III)(ppy)2(dtbpy) | Ir(III)*/Ir(II) | 0.66 V | Ir(III)*/Ir(IV) | -0.96 V |
To overcome these limitations, Wenger and coworkers recently reviewed multi-photon synthetic concepts and approaches looking to exploit multi-excitation processes for synthetic applications (Ref 1). Spectroscopists routinely study multi-photon reactions with expensive pulsed lasers, short-lived excited intermediates and low micromolar concentrations. However, for synthetic purposes the utility of multiphoton excitation is a recent development with the availability of high-powered LEDs.
Consecutive Photo-Excitation as a Multi-Photon Approach to Synthetic Photochemistry
The simplest scenario to utilize two photons of energy involves consecutive excitations to produce a highly reducing species (Figure 1). The ground state photocatalyst (PC) absorbs the first photon of light generating an excited triplet state. If the resulting excited photocatalyst has a sufficient lifetime and a suitable excitation band, a second photon excitation can result in a higher excited state with ultimate release of the superreductant hydrated electron (e·-aq (-2.9 V vs. NHE). The system can be made catalytic in the presence of a sacrificial donor to return the photocatalyst.
Figure 1: Consecutive photoexcitation of photocatalyst
Wenger demonstrated the generation of hydrated electrons in two recent examples (Ref 3,4). Using the photocatalyst Ir(sppy)3 (Figure 2A), triethanolamine (“TEAO”) and a blue continuous wave laser (447 nm) afforded conditions suitable for several powerful reductions (Ref 3). The consecutive two photon excitation mechanism was demonstrated with a pulsed laser system. The initial laser pulse generates a triplet excited Ir(sppy)3 species with a lifetime of 1.6 μs and a strong absorbance between 460 nm and 570 nm. The second laser pulse at 532 nm promotes a higher excited state with photoionization to give the hydrated election. Both ground and excited state excitation of Ir(sppy)3 occurred with a single continuous wave 447 nm diode laser. This powerful reduction with a low energy (inexpensive) laser and water-soluble catalyst represents an opportunity for the degradation of pollutants. For example, the CF3 functional group is a common inert group in many pharmaceuticals and pesticides while the benzylammonium cation is a common wastewater pollutant (Figure 2B).
Figure 2: A). Ir(sspy)3 catalyst B.) Applications of hydrated electron reductions
With the same system, Wenger and coworkers demonstrated reaction control through the modification of the light source intensity(Ref 4). At low intensity, the catalyst acts as a photoredox catalyst (E1/2 = -1.6 V) for one electron reduction. At higher intensity light focused with a lens, the two-photon process generates the superreductant e·-aq (E1/2 = -2.9 V) nearly doubling the available redox energy compared to the one photon system. This selectively was demonstrated with the dehalogenation of 4-bromo-2-chloro-5-fluorobenzoic acid (Figure 3). At low intensity light, the primary pathway involves reductive activation of the weaker C-Br bond. Under the two-photon process, the reduction potential was sufficient for activation of the C-Cl bond. Neither system activated the strongest C-F bond.
Figure 3. Control over light intensity allows selectivity in the reaction. Irridiation of Ir(sspy) catalyst, TEOA in water.
Consecutive Photoinduced Electron Transfer (ConPET) as a Multi-Photon Approach to Synthetic Photochemistry
In an alternate approach, classified as a Consecutive Photoinduced Electron Transfer (ConPET), the two photon excitation steps are separated by reduction of the photocatalyst (Figure 4). Here, the first photon results in an excited photocatalyst, which upon reduction by a sacrificial reductant generates a photoactive radical anion species suitable for excitation with a second electron. It is then the radical anion of the PC that is excited by the second photon generating the excited triplet photocatalyst photoionizing to the solvated electron. The scheme was first demonstrated by Konig and coworkers (Ref 5) using the PDI photocatalyst (Figure 5A) and blue LEDs for the activation of the carbon-halogen bonds (Figure 5B). Konig et. al. use this system to activate aromatic chlorides demonstrating the dehalogenation and successful cross-coupling with multiple coupling agents. Alternative approaches have used an electrode to replace the sacrificial donor in this scheme (Ref 6).
Figure 4: Scheme for Consecutive Photoinduced Electron Transfer (ConPET)
Figure 5: A.) Structure of photocatalyst perylene diimide (PDI), B.) ConPET dechlorination
Photocatalytic Birch Reductionas a Multi-Photon Approach to Synthetic Photochemistry
Recently, Miyake reported the Birch reduction using LEDs and new organic photoredox catalysts (Ref 7). By harnessing the power of a multi-photon catalysis, Miyake and coworkers have successfully demonstrated this difficult reaction with mild, metal-free visible light driven conditions. The Birch reduction is a powerful classical reaction that requires harsh reagents. Formally, the simplest example is the 2 e–/2H+ reduction of benzene (Ered <-3.42 V vs. SCE) to 1,4-cyclohexadiene (Figure 6). One of the most difficult and dangerous reductions in use, the typical Birch reduction uses solvated electrons as the reductant (usually involving lithium or sodium metal, cryogenic temperature and liquid ammonia). Due to its utility, many advances have been undertaken to attempt to increase the safety.
Figure 6: Classical Birch reduction
Miyake synthesized a series of organic catalysts benzo[ghi]perylene monoimides (BPIs) as photocatalysts. These catalysts are calculated to have significantly more negative reduction potentials than observed for PDI. After extensive method development, the authors report the reduction of a wide range of aromatic substrates (Figure 7). Using 405 nm LEDs, NMe4OH as the electron source, and low concentrations of the BPI catalyst the birch reduction proceeds with high yields and selectivity. Scaling the reaction to 10 mmol proceeded without the loss of yield, with several examples greater than 70%. For this work, the reduction of the photocatalyst acts as the first step, with the formation of photocatalyst-donor complex using either OH- of F- (Figure 8). Two successive photoinduced electron transfer steps generate the e·-aq as the strong reductant for the Birch reduction. The persistent radical nature of PC·- affords a species which can be excited with LEDs rather than laser irradiation. Within the report, Miyake describes extensive mechanistic analysis demonstrating each state of the photocatalyst during the catalytic cycle. This extensive analysis should prove valuable for expanding the substrate scope of this mild and incredibly useful reduction.
Figure 7: Visible light photocatalyzed Birch reduction
Figure 8: Modified ConPET mechanism
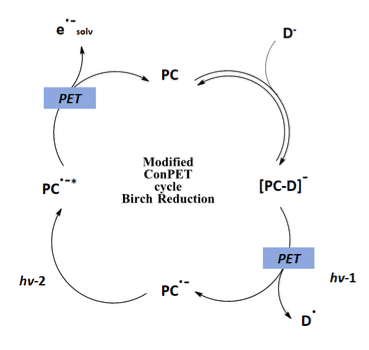
Adapted from Ref 7
Summary:
By utilizing the power of multiple photon excitations, the available chemistry for visible light photochemistry can be greatly expanded. Here we have discussed two approaches to multi-photon excitation, with successive excitation steps. Alternatively, Wenger discusses additional complex systems including separation of the excitation steps, multiple photo-catalysts and triplet-triplet fusion. For a detailed analysis, please read this exciting review (Ref 1).
Explore our previous discussions on Electron Donor Acceptor Complexes and Near Infrared (NIR) applications in photochemistry.
References:
- Felix Glaser, Christoph Kerzig, and Oliver S. Wenger, “Multi-Photon Excitation in Photoredox Catalysis: Concepts, Applications, Methods” Angew. Chem. Int. Ed., 2020, 59(26), 10266-10284. https://doi.org/10.1002/anie.201915762
- Merck Catalysis Center: http://chemlabs.princeton.edu/macmillan/wp-content/uploads/sites/6/Merck-Photocatalysis-Chart.pdf
- C. Kerzig, X. Guo, O. S. Wenger, “Unexpected Hydrated Electron Source for Preparative Visible-Light Driven Photoredox Catalysis” J. Am. Chem. Soc. 2019, 141, 2122- 2127. https://pubs.acs.org/doi/10.1021/jacs.8b12223
- C. Kerzig, O. S. Wenger, “Reactivity control of a photocatalytic system by changing the light intensity” Chem. Sci. 2019, 10, 11023-11029. https://pubs.rsc.org/en/content/articlehtml/2019/sc/c9sc04584h
- Ghosh, I.; Ghosh, T.; Bardagi, J. I.; König, B. “Reduction of aryl halides by consecutive visible light-induced electron transfer processes”. Science 2014, 346, 725-728. https://science.sciencemag.org/content/346/6210/725
- Cowper, N. G. W.; Chernowsky, C. P.; Williams, O. P.; Wickens, Z. K. Potent Reductants via Electron-Primed Photoredox Catalysis: Unlocking Aryl Chlorides for Radical Coupling. J. Am. Chem. Soc., 2020, 142, 2093-2099. https://pubs.acs.org/doi/abs/10.1021/jacs.9b12328
- Justin P. Cole, Dian-Feng Chen, Max Kudisch, Ryan M. Pearson, Chern-Hooi Lim, and Garret M. Miyake, “Organocatalyzed Birch Reduction Driven by Visible Light, J. Am. Chem. Soc, 2020, 142, 13573-13581. https://pubs.acs.org/doi/abs/10.1021/jacs.0c05899